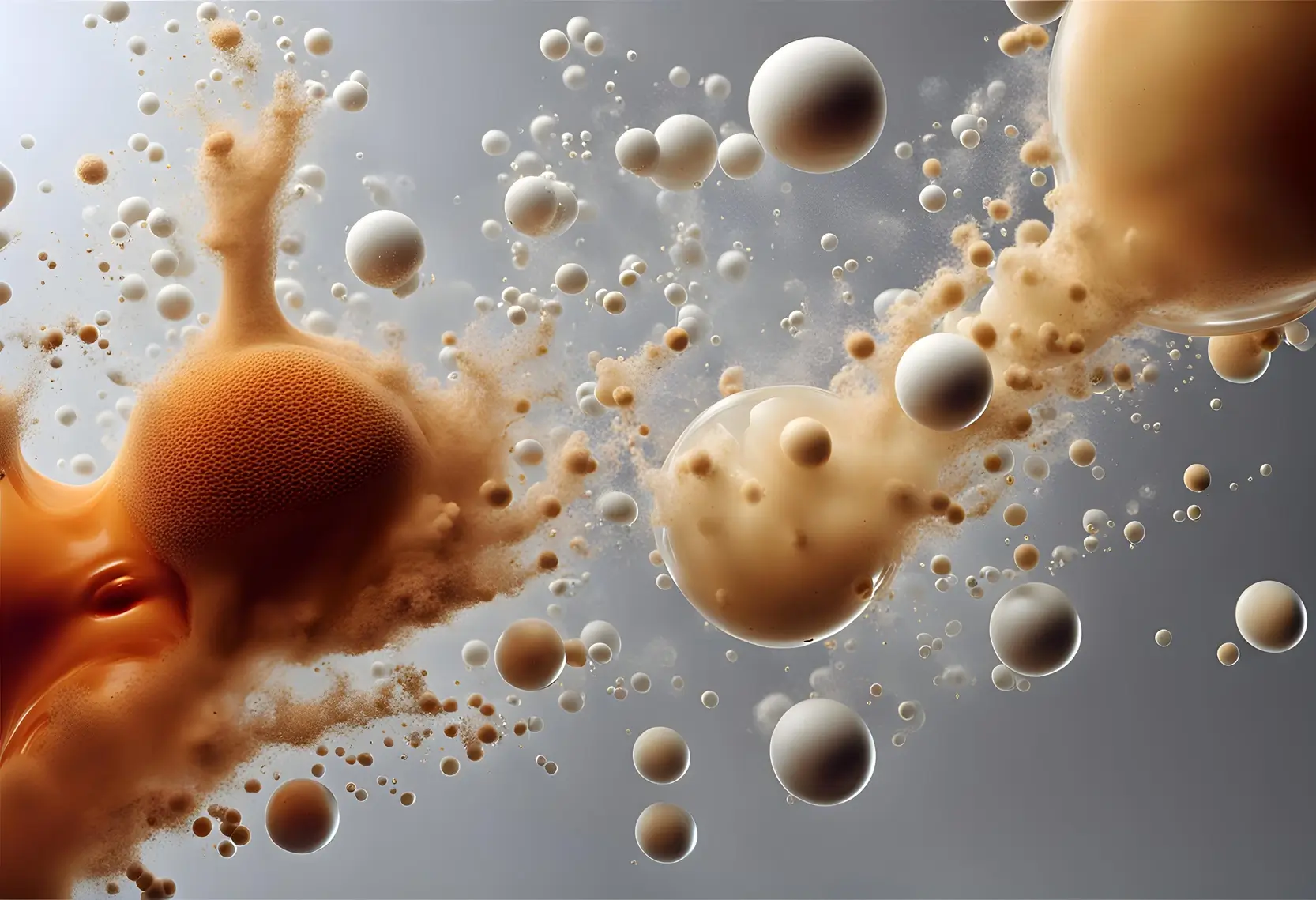
The production of powders and particulate materials in gases and liquids encompasses a variety of techniques beyond traditional methods like spray drying, precipitation, and crystallization. Emerging technologies, notably microfluidics, play an important role in this domain, offering unparalleled precision and control over particle formation and manipulation. Through microfluidics, researchers can tailor the properties of powders and particulate materials with superior detail, including size, shape, and composition. This advancement opens up new perspectives in materials science, facilitating the creation of custom-formulated materials with tailored functionalities and applications.
Beginnings
The history of microfluidics dates back to the mid-20th century when advancements in microfabrication techniques, such as photolithography and thin-film deposition, enabled the precise manipulation of fluids at the microscale. Coined in the 1980s, the term “microfluidics” encapsulated this emerging field, which aimed to miniaturize fluidic systems for various applications. The concept of “lab-on-a-chip” emerged in the 1990s, envisioning complete analytical systems integrated onto a single microchip. Pioneering research during this period laid the foundation for key microfluidic techniques, including DNA analysis, cell manipulation, and droplet-based assays. By the 2000s, microfluidics had transitioned from
academic research to commercialization, with standardized platforms offered for diverse applications.
This period witnessed the integration of microfluidics with other technologies, such as microelectronics and nanotechnology, leading to more complex and versatile systems.
Ongoing advancements in materials science and manufacturing techniques continue to expand the capabilities of microfluidics, driving innovation in fields ranging from biotechnology to personalized medicine. Overall, the history of microfluidics reflects a journey of continuous innovation and interdisciplinary collaboration, culminating in its status as a cornerstone of modern technology.
Ongoing advancements in materials science and manufacturing techniques continue to expand the capabilities of microfluidics, driving innovation in fields ranging from biotechnology to personalized medicine. Overall, the history of microfluidics reflects a journey of continuous innovation and interdisciplinary collaboration, culminating in its status as a cornerstone of modern technology.
Microfluidics for Particle Manipulation
The very foundation laid by pioneering advancements in microfabrication techniques, as discussed earlier, paved the way for the precise control and manipulation of fluids at the microscale. This control, once a futuristic dream, has become the cornerstone of microfluidic particle manipulation, enabling the creation of particles with tailored properties and functionalities unimaginable before. Microfluidics involves the manipulation of fluids on a microscale, typically in channels with dimensions ranging from tens to hundreds of microns. This technology has shown great potential in the production and manipulation of particles, offering advantages such as reduced manufacturing costs, lower reagent consumption, and increased device efficiency. Various fabrication methods for microfluidic devices, including low-volume and high-volume production techniques, have been developed to support the integration of microfluidics in particle manipulation processes.
Fluid Dynamics at the Microscale
Fluid dynamics at the microscale present unique phenomena compared to macroscale fluid dynamics. This distinction arises from the dominance of surface effects and the low Reynolds numbers characteristic of microfluidic systems. In macroscale fluid dynamics, inertial forces are significant, leading to turbulence and complex flow patterns. However, in microfluidics, viscous forces predominate due to the small dimensions involved, resulting in laminar flow and diffusion-driven mixing. Laminar flow, prevalent in microfluidic channels, is characterized by fluid streams moving in parallel layers with minimal mixing between them. This orderly flow behavior is attributed to the low Reynolds numbers in microfluidic systems, where inertial forces are negligible compared to viscous forces.
As a consequence, the fluid flows smoothly, maintaining distinct boundaries between layers, which is
advantageous for applications requiring precise fluid control. The dominance of viscous forces in microfluidics allows for precise manipulation of fluid streams and minimizes energy dissipation. Furthermore, the low Reynolds number in microfluidic systems indicates the supremacy of viscous forces and the absence of turbulent flow regimes, facilitating predictable fluid behavior. Surface effects become pronounced in microfluidic channels due to the large surface area-to-volume ratio. Capillary action, governed by intermolecular forces such as cohesion and adhesion, influences fluid behavior at the microscale. This phenomenon is exploited in microfluidic devices for tasks like sample transport, droplet formation, and surface patterning, enhancing the versatility and functionality of microfluidic systems.
While laminar flow and diffusion-dominated mixing offer advantages in microfluidic applications, they also pose challenges, particularly in achieving efficient mixing and mass transfer. One challenge posed by laminar flow and diffusion-dominated mixing is the efficient mixing and mass transfer required for certain applications. Furthermore, gas-liquid interactions play a pivotal role in microfluidic systems, influencing processes such as bubble formation and droplet generation. In microchannels, gases can be introduced either as discrete bubbles or dispersed within a continuous liquid phase. The dynamics of gas-liquid interactions are influenced by parameters such as flow rates, channel geometries, and surface properties. For instance, in biochemical assays or chemical reactions, achieving rapid and uniform mixing of reagents within microfluidic channels is essential for accurate results. Researchers have tackled this challenge through innovative designs, such as passive mixers employing chaotic advection or active mixing strategies using external stimuli like acoustic waves or magnetic fields. Additionally, advancements in numerical simulations and experimental techniques contribute to a deeper understanding of fluid dynamics at the microscale, driving continuous improvement and innovation in microfluidic technology.
Fabrication Techniques
Various fabrication techniques are employed in the creation of microfluidic devices, each geared towards achieving precise features and dimensions necessary for optimal performance. Microfluidic devices are often fabricated using materials such as polymers (e.g., polydimethylsiloxane or PDMS), glass, and silicon. PDMS is a popular choice due to its biocompatibility, transparency, and ease of fabrication using soft lithography techniques. Glass and silicon offer advantages in terms of optical transparency, mechanical rigidity, and compatibility with microfabrication processes such as photolithography and etching.
Researchers address these challenges through innovative designs, such as passive and active mixing strategies, to enhance fluid mixing and reaction kinetics in microfluidic devices. Additionally, advancements in numerical simulations and experimental techniques contribute to a deeper understanding of fluid dynamics at the microscale, driving continuous improvement and innovation in microfluidic technology. As microfluidics continues to evolve, future research directions may focus on exploring complex fluid phenomena, such as multiphase flows and non-Newtonian behavior, in microscale systems. Interdisciplinary collaborations between fluid dynamics, materials science, and engineering disciplines can lead to the development of novel microfluidic platforms with enhanced functionalities for diverse applications, including healthcare, environmental monitoring, and advanced manufacturing.
Photolithography is a foundational step in microfluidic device fabrication. It involves depositing a photosensitive material, such as a photoresist, onto a substrate and exposing it to UV light through a photomask. This process creates a pattern defining the desired channel geometry. Subsequent development and etching steps transfer this pattern onto the substrate, forming the basis of the microfluidic structure. Soft Lithography builds upon photolithography by utilizing the patterned master mold created through photolithography. PDMS is cast onto this mold, forming a replica with microfluidic channels. The PDMS replica is then carefully bonded to a substrate, resulting in the formation of the final microfluidic device. This technique allows for precise control over channel dimensions and layout, essential for fluid manipulation and interaction. Etching, whether through wet or dry processes, further refines the features of microfluidic channels. By selectively removing material from the substrate, intricate channel geometries are defined. Precise control over etchant composition and processing parameters ensures the desired channel geometry is achieved, enhancing the performance of the microfluidic device.
These techniques are not mutually exclusive and can be combined to create complex microfluidic devices with tailored functionalities. For instance, photolithography can be used to define the overall channel layout, while soft lithography is employed to create specific features within those channels. Additionally, etching can be utilized to further refine channel dimensions or create additional structures within the device. By integrating these techniques, researchers can design and fabricate microfluidic devices tailored to their specific applications.
Aside from the established techniques of photolithography, soft lithography, and etching, additive
manufacturing methods such as stereolithography or inkjet printing offer new avenues for microfluidic device fabrication. While traditional techniques excel in defining overall channel layouts and refining channel features, additive manufacturing provides a direct means of producing microfluidic structures layer-by-layer. This approach enables rapid prototyping and customization of microfluidic devices, building on innovation and experimentation in fluidic system design. By seamlessly integrating these diverse fabrication techniques, researchers can harness the full potential of microfluidics to create tailored devices suited to a myriad of applications.
Microfluidic systems
Microfluidic systems serve as platforms for a diverse array of chemical reactions and interactions, owing to their precise control over fluid flow and mixing. Microreactors within microfluidic devices enable the rapid and efficient synthesis of organic and inorganic compounds. By finely tuning reaction conditions such as temperature, pressure, and reactant concentrations, researchers can achieve high yields and selectivity in chemical synthesis processes. Microfluidic devices are invaluable tools for high-throughput screening of biological samples, enabling miniaturized assays for applications including drug discovery and diagnostics. By integrating various functionalities such as sample preparation mixing, and detection within a single device, microfluidics streamlines assay workflows and conserves precious reagents and samples. Microfluidic platforms facilitate precise manipulation and separation of analytes, enhancing the performance of analytical techniques such as chromatography, electrophoresis, and mass
spectrometry. Through miniaturization and integration of sample handling and detection components, microfluidic systems offer improved sensitivity, resolution, and throughput in chemical analysis.
Microfluidic systems serve as versatile platforms for a diverse array of chemical reactions and interactions, facilitated by their precise control over fluid flow and mixing. Additionally, their integration of sample handling and detection components within a single device streamlines assay workflows, further enhancing their utility in various applications. Microreactors within microfluidic devices enable the rapid and efficient synthesis of organic and inorganic compounds. By finely tuning reaction conditions such as temperature, pressure, and reactant concentrations, researchers can achieve high yields and selectivity in chemical synthesis processes. Microfluidic devices are invaluable tools for high-throughput screening of biological samples, enabling miniaturized assays for applications including drug discovery and diagnostics.
Microfluidic platforms have revolutionized drug delivery by offering precise control over drug formulations and release kinetics. For instance, researchers have developed microfluidic-based devices capable of on-demand drug release triggered by external stimuli, such as pH or temperature changes, allowing for personalized treatment regimens tailored to individual patient needs. In biomedical diagnostics, microfluidic-based lab-on-a-chip devices have enabled rapid and sensitive detection of various diseases, including infectious pathogens and cancer biomarkers, with the potential for point-of-care testing in resource-limited settings. Moreover, in tissue engineering, microfluidic systems have facilitated the fabrication of intricate multicellular constructs with spatial control over cell organization and nutrient gradients, mimicking the complexity of native tissues for applications in regenerative medicine. By integrating various functionalities such as sample preparation, mixing, and detection within a single device, microfluidics streamlines assay workflows and conserves precious reagents and samples.
By mimicking physiological conditions and enabling real-time monitoring of cellular responses, microfluidic platforms yield insights into cellular behavior and function. These capabilities find applications in fields ranging from fundamental cell biology research to drug screening and personalized medicine.
Advanced Applications in Biomedical Engineering
Microfluidic platforms have transformed drug delivery systems through precise control over drug formulations, release kinetics, and targeting strategies. By enabling personalized treatment regimens tailored to individual patient needs, these platforms have revolutionized the field of drug delivery. One notable application is the development of microfluidic-based drug-delivery devices for personalized medicine. These devices allow for the encapsulation of drugs within biocompatible carriers, such as nanoparticles or liposomes, tailored to individual patient needs. Additionally, microfluidic systems facilitate the continuous production of drug-loaded particles with controlled size and morphology, enhancing drug efficacy and reducing side effects.
Biomedical Diagnostics
Microfluidics has significantly advanced biomedical diagnostics by providing miniaturized, portable, and cost-effective solutions for disease detection and monitoring. Lab-on-a-chip devices, powered by microfluidic technology, integrate multiple assay steps, including sample preparation, reaction, and detection, onto a single chip. This integration enables rapid and automated analysis of biological samples, making it ideal for point-of-care diagnostics. For example, microfluidic-based systems have been developed for detecting infectious diseases, cancer biomarkers, and genetic mutations with high sensitivity and specificity. Moreover, the scalability and multiplexing capabilities of microfluidic platforms offer opportunities for high-throughput screening and personalized medicine applications.
Emerging Technologies in Microfluidics
Microfluidics continues to evolve with the emergence of new technologies and techniques that enhance particle production and manipulation capabilities. These emerging technologies hold great promise for various applications, including additive manufacturing and biomedical research.
Droplet-based microfluidics enables the generation and manipulation of discrete liquid droplets within microchannels. This technology has gained widespread attention for its versatility in creating monodisperse emulsions, encapsulating cells or molecules and performing high-throughput assays. In the context of particle production, droplet-based microfluidics offers precise control over particle size,
composition, and functionality. Researchers are exploring its applications in drug delivery, where droplets serve as carriers for controlled release formulations, as well as in tissue engineering, where droplet microfluidics enables the fabrication of complex multicellular constructs.
Acoustofluidics integrates acoustic waves with microfluidic systems to manipulate particles and cells with unparalleled precision. By harnessing acoustic forces, researchers can manipulate particles based on their size, density, and compressibility, offering a non-contact and label-free approach to particle manipulation. Acoustofluidic devices can achieve tasks such as sorting, trapping, and concentrating
particles with high efficiency and throughput. In particle production, acoustofluidics enables the generation of uniform particles and the removal of contaminants, leading to improved product quality and process efficiency. This technology holds promise for applications in drug discovery, cell therapy, and environmental monitoring, where precise control over particles is essential for success.
Conclusion
In the ever-expanding domain of microfluidics, the possibilities are as vast as our imagination. The realm of microfluidics stands as a beacon of innovation and limitless potential, offering boundless opportunities for exploration and advancement. As research discovers more intricacies of fluid dynamics at the microscale and pushes the boundaries of fabrication techniques, we unlock new areas of possibility in particle manipulation, chemical synthesis, biomedical engineering, and beyond.
Looking ahead, at the emerging technologies like droplet-based microfluidics and acoustofluidics redefining the landscape of particle production and manipulation. These cutting-edge approaches are paving the way for increased precision and efficiency in drug delivery, tissue engineering, and environmental monitoring, revolutionizing how we approach healthcare, biotechnology, and beyond.